The Quantum Cascade Laser
This section is dedicated to the Quantum Cascade Lasers and current topics in research and applications. It links to resources beyond the scope of the original research of the Faist group.
More about Quantum Cascade Lasers
Terahertz Quantum Cascade Lasers
The Quantum Cascade Laser
Because of their small size (typically the size of a pin's head), high reliability and ease of use, conventional semiconductor diode lasers play a dominant role in telecommunications and consumer electronics in applications such as optical fiber communications and compact disk (CD) players. In these lasers, the light originates from the recombination of negative and positive charges (electrons and holes) across the energy gap existing between the conduction and valence band of the crystal. The energy separation between the two bands thus determines the lasing wavelength. This separation is called bandgap and, in general, is dependent on which material had been used.
Instead, the Quantum Cascade laser (QCL) is a semiconductor laser involving only one type of carriers and which is based on two fundamental phenomena of quantum mechanics, namely tunneling and quantum confinement. Although the basic concept was proposed as early as 1971, it took more than twenty years before an actual device was demonstrated, in 1994 [1]. Whereas in normal diode lasers the photon emission is based on electron-hole recombination across the bandgap, the QCL is based on a completely different approach. In a QCL, the photon emission is obtained by exploiting so-called intersubband transitions, where electrons are scattered between bound states created by quantum confinement in ultrathin (<50 nm) alternating layers of semiconductors materials.
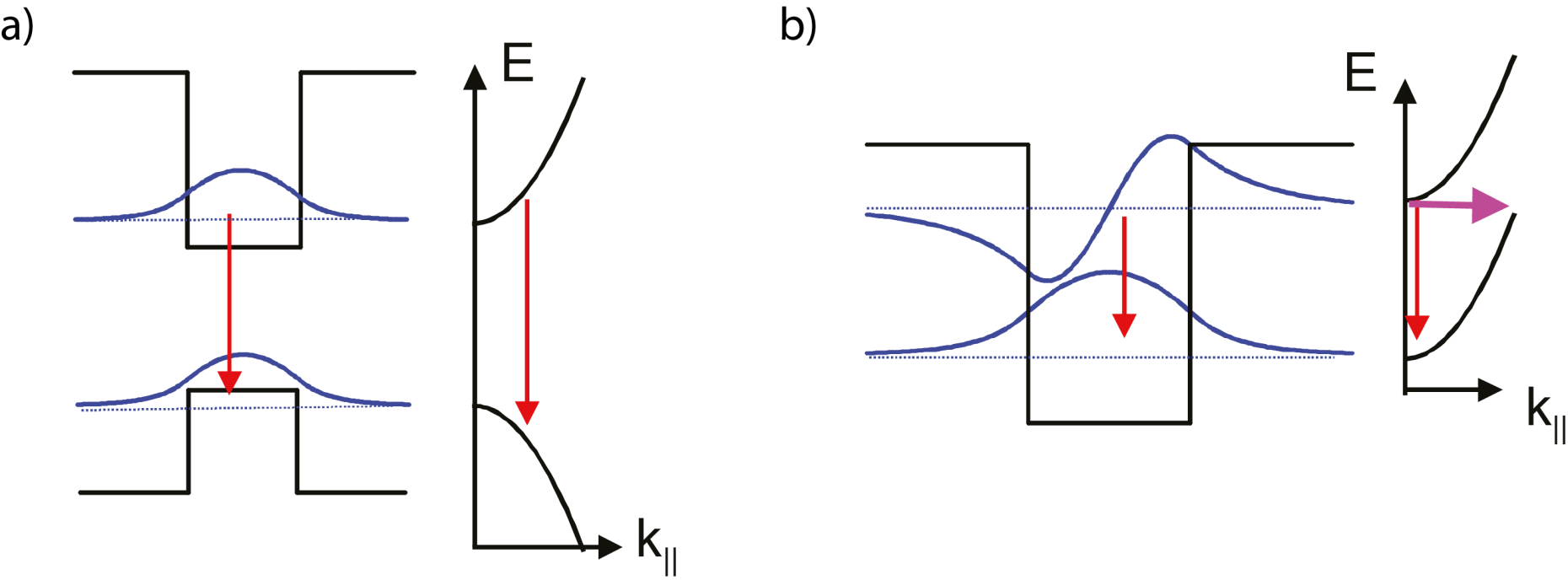
Since these ultrathin layers, called quantum wells, have a size comparable to the electron's De Broglie wavelength, they quantize the electrons energy levels in the direction perpendicular to the plane of the layers. When inside a quantum well, an electron can jump from one state to the other by discrete steps, sometimes emitting photons. The spacing between the energy levels depends on the width of the well, and increases as the well size is decreased. As a consequence, the emission wavelength depends now on the layer thicknesses and not anymore on the bandgap of the constituent materials, leading to a broad frequency flexibility of this device. This has indeed allowed the manufacturing of lasers with emission wavelengths ranging from 2.7 to 250 micrometers using combinations of commonly used III-V semicnductors as InGaAs/AlInAs/InP, GaAs/AlGaAs and InAs/AlSb.
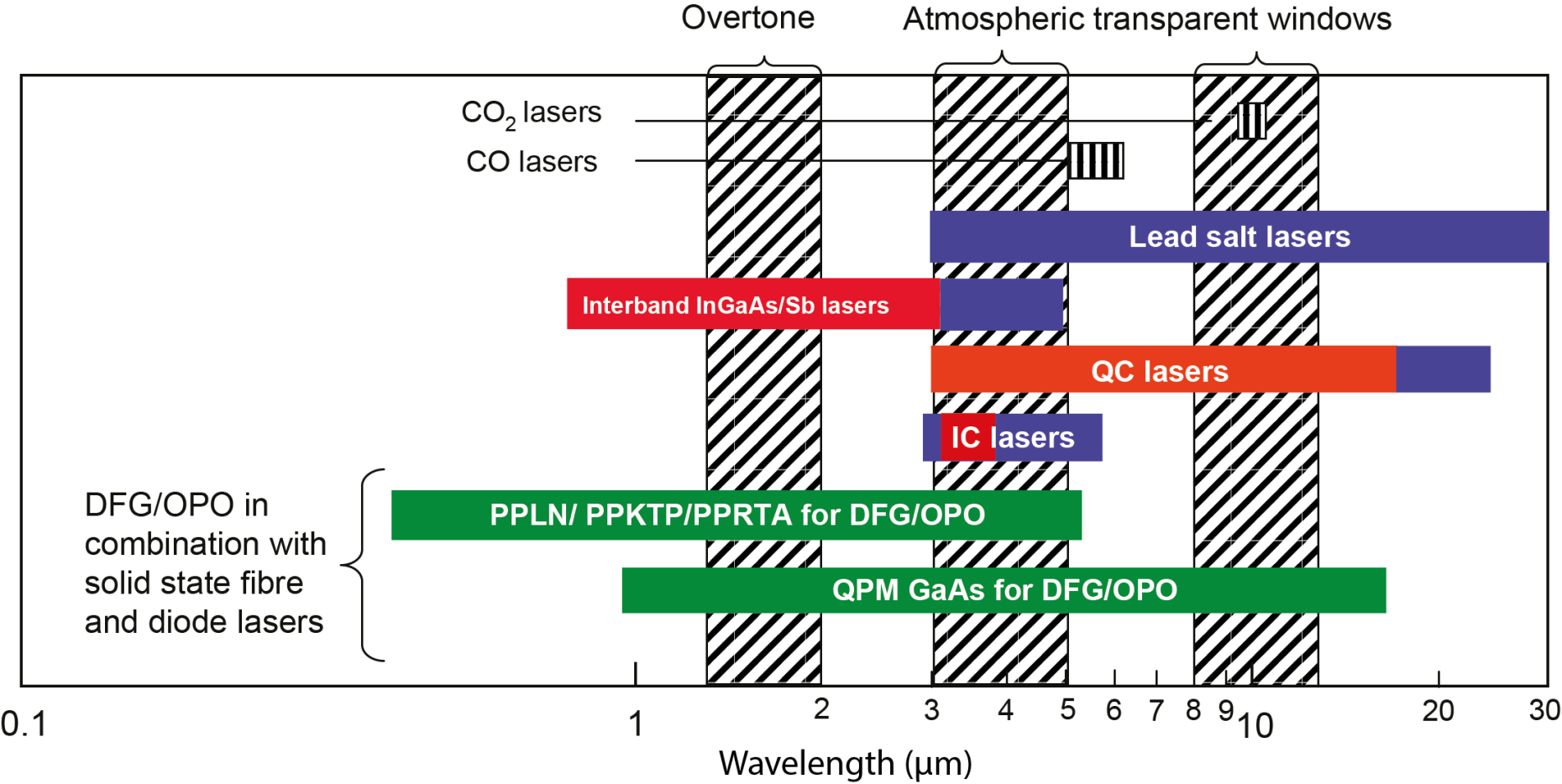
Of course, having an intersubband transition is still far away from obtaining a working QCL. A QCL is way more sophisticated and one needs quantum engineering in order to achieve all the requirements needed to create a Quantum Cascade Laser. These are:
- Electrical stability at the operating point
- Population inversion and gain obtained by electron lifetime engineering
- Low loss optical resonator to match gain value and allow laser oscillation
The following figure shows the quantum well schematic that allowed to achieve lasing emission in the first QCL demonstration in 1994 at Bell Labs by J.Faist.
A QCL is composed by several periods of an elemental structure based on two region: the active region and the relaxation/injection region. The active region can be seen as a standard three level system where each level is a confined state. The lifetime of the transition from level 3 to 2 has to be longer than lifetime of the electrons in level 2 if one wants to achieve population inversion, an essential condition for obtaining gain. Following the active region, comes the injection/relaxation region, whose purpose is to transport the electrons to the following active region down the cascade.
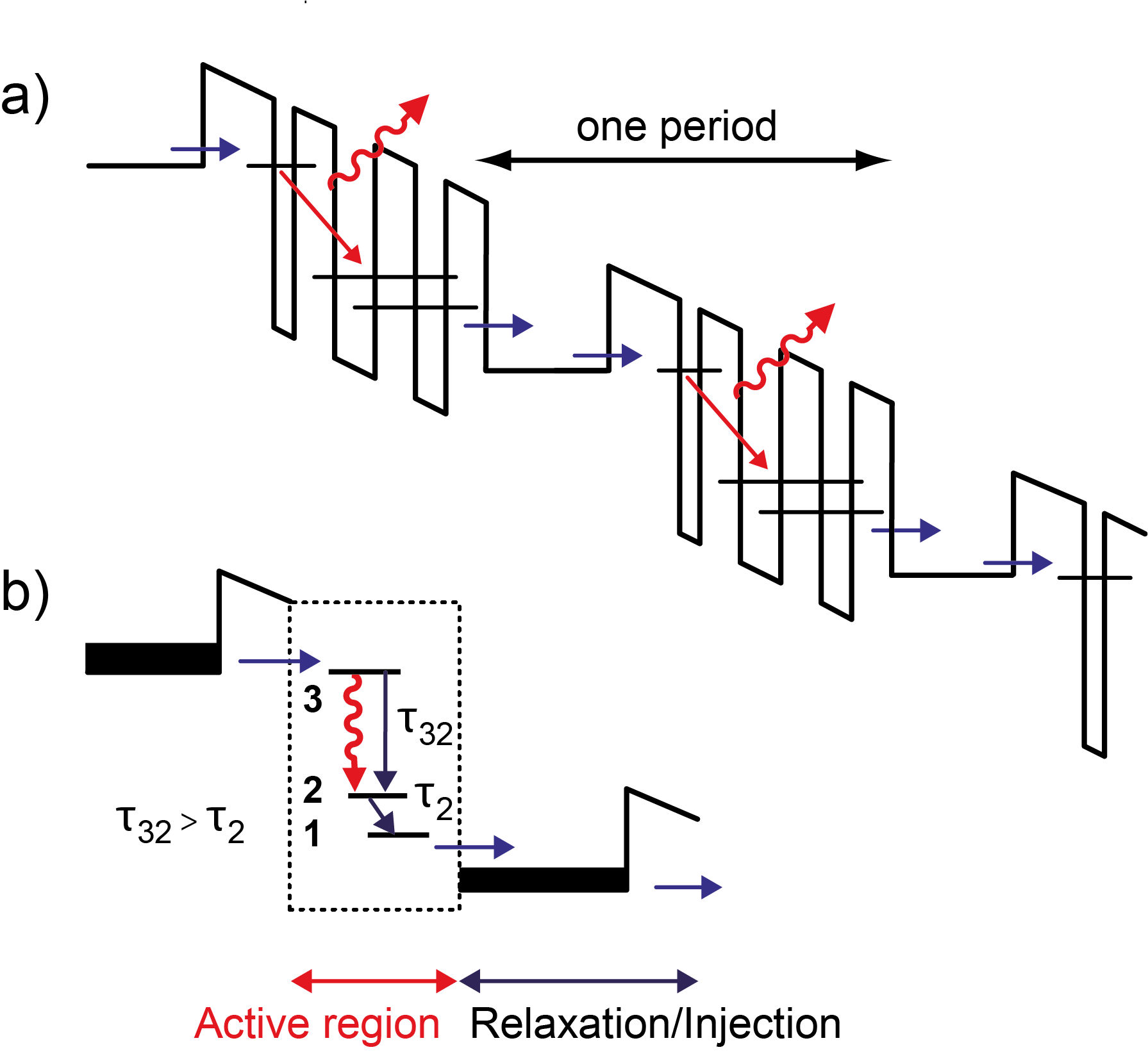
The operating principle of the quantum cascade laser can be summarized as follows:
- under the right applied bias, an electron will be injected via resonant tunneling in the upper state of the active region and relax to the lower state eventually emitting a photon
- from here the electron will be transported through the relaxation region and then injected into the upper state of the following period where it will again be able to emit a photon
- if the population inversion is ensured, as the current is increased gain will match the losses and the device will reach threshold, converting the injected electrons into photons by stimulated emission. The emitted power will depend linearly on the injected current.
In this way, each electron involved in the process can actually generate at most N photons, with N number of periods of the structure, when cascading across the structure.
Compared to conventional (interband) lasers, the quantum cascade laser differs by the following important points:
- As mentioned above, the same semiconductor material system whose technology is very well mastered can be used to manufacture lasers operating across the whole mid-infrared and THz ranges
- It is based on a cascade of identical stages (typically 20-50), allowing one electron to emit many photons, emitting more optical power (electron recycling)
- Due to its unipolar nature it does not suffer from surface recombination
- Due to ultrafast electron lifetimes, QCLs are intrinsically high speed devices and can be modulated to extremely high frequencies.
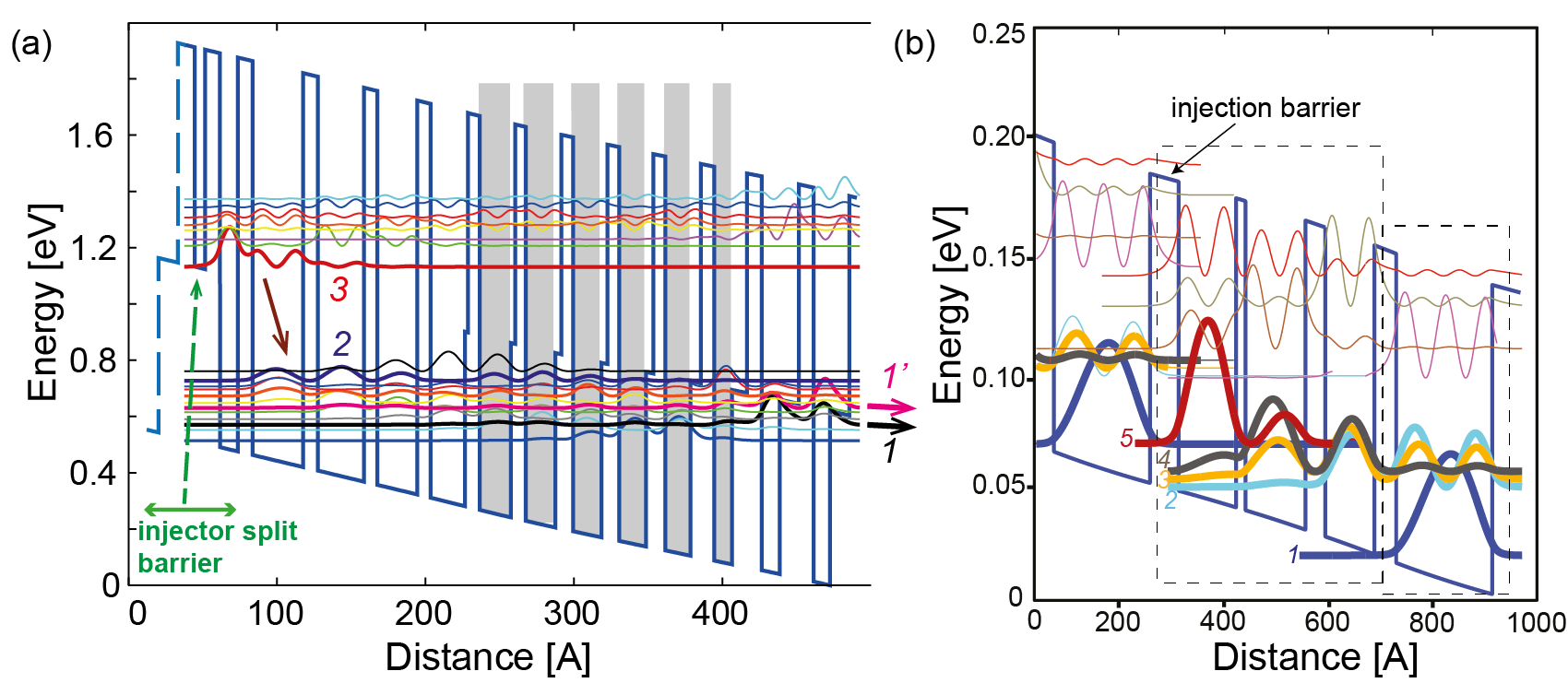
Today, QCLs are finding their space into modern technology due to their compactness, high power and peculiar frequency range. Despite the progress, though, several challenges are still open and pursued by the scientific community:
- Room Temperature operation for THz QCLs: although mid-infrared QCLs are extremely robust and can operate at temperature higher than 20 Celsius degrees, the THz QCLs struggle to lase above 200 K. This is due to an extreme sensitivity to phonons population inside the system, which hinders radiative emission at relatively high temperature. Solution are still being studied in order to obtain a room temperature THz QCL and consequently to achieve a huge technological upgrade
- New material systems: as said above, the QCL is freed from the choice of the bandgap and it can be based on several material systems. A huge effort is always spent on researching new possible material systems, in particular Si/SiGe, which would then enable the possibility to integrate coherent light sources into Si photonic devices [4]
- Quantum cascade frequency combs: recently our lab demonstrated that broadband quantum cascade lasers display phase coherence between the modes due to an ultrafast internal third order non linearity [5]. Such lasers can be then used as frequency combs. This can have a tremendous impact on Mid-IR laser spectroscopy leveraging on the very small footprint of the QCL and its unique characteristics of spectral agility, rapidity and high resolution. THz devices are particularly promising for ultra-wide bandwidth as our group already demonstrated octave-spanning emission from heterogeneous QCL [6]
Further details and introductory talks can be found in the links at the bottom of this page. If you want to get deep into research, instead, look at all the things we are doing in our labs.
References
[1] J. Faist, F. Capasso, D. L. Sivco, C. Sirtori, A. L. Hutchinson, and A. Y. Cho " Quantum Cascade Laser", Science, 264, 553, (1994)
[2] J. Faist, "Quantum cascade lasers", Oxford (2014).
[3] M. I. Amanti, et al. "Bound-to-continuum terahertz quantum cascade laser with a single-quantum-well phonon extraction/injection stage", New J. Phys. 11, (2009)
[5] A. Hugi, et al. "Mid-infrared frequency comb based on a quantum cascade laser", Nature 492, 229–33 (2012).
[6] M. Rösch, et al. "Octave-spanning semiconductor laser", Nat. Photonics 9, 42–47 (2014).
More about Quantum Cascade Lasers
2015 review article on QCLs and applications:
external page Quantum cascade lasers: 20 years of challenges
Miriam Serena Vitiello, Giacomo Scalari, Benjamin Williams, and Paolo De Natale
Optics Express, Vol. 23, Issue 4, pp. 5167-5182 (2015)
Tutorial presentations from the "20 Years of QCL" conference in Zurich, 2014:
QCL's: How/why it happened and future outlook
Capasso Federico
20 years of quantum cascade lasers: key steps and future promises
Jérôme Faist
Quantum cascade lasers: our initial concept and some of recent theoretical developments
Kazarinov Rudolf F ; Suris Robert A
How Molecular Beam Epitaxy (MBE) was invented and how MBE is used for Quantum Cascade Lasers (QCL)
Al Y. Cho
Terahertz Quantum Cascade Lasers
2007 Review article on THz QCLs:
external page Terahertz quantum-cascade lasers
Benjamin S. Williams
Nature Photonics 1, 517 - 525 (2007)
Tutorial presentations from the "20 Years of QCL" conference in Zurich, 2014:
The terahertz QCL: an unexpected journey
Alessandro Tredicucci
Quantum Cascade Laser Frequency Combs
2016 Review article:
external page Quantum Cascade Laser Frequency Combs
Jérôme Faist, Gustavo Villares, Giacomo Scalari, Markus Rösch, Christopher Bonzon,
Andreas Hugi, and Mattias Beck
Nanophotonics, Vol 5, 2 (2016)
Tutorial presentations from the "20 Years of QCL" conference in Zurich, 2014:
Quantum Cascade Laser frequency Combs
Andreas Hugi
THz frequency combs, light amplifiers, and antenna-enhanced emitters
Qing Hu
Quantum Cascade Laser Applications
Review article including applications
external page Quantum cascade lasers: 20 years of challenges
Miriam Serena Vitiello, Giacomo Scalari, Benjamin Williams, and Paolo De Natale
Optics Express, Vol. 23, Issue 4, pp. 5167-5182 (2015)
Tutorial presentations from the "20 Years of QCL" conference in Zurich, 2014:
Mid-infrared semiconductor laser based trace gas technologies: recent advances and applications
Tittel Frank
Commercialization and Applications of Quantum Cascade Lasers and Sensors
Timothy Day
Recent commercial efforts in and for applications connected to the Faist lab
external page Ultrafast and high resolution Dual-Comb spectrometers IRsweep
external page Demo of first IRsweep Dual-Comb QCL spectrometer
external page Quantum Cascade Laser devices Alpes Lasers